Keynote and Invited Talks
Keynote and Invited Talks
Keynote
Possibility of Mass Diffusion Control: Effect of Pore Size of Separated Membrane
Professor Atsuki Komiya
Institute of Fluid Science, Tohoku University
A better understanding of protein transport phenomena is one of the essential key factors in medicine and the biochemical industry. This study focuses on the precise control of protein mass diffusion by using a membrane having macropore patterning. Experimentally the mass flux through the membrane was measured and the relationship between hindered mass diffusion flux and macropore diameter on the membrane was evaluated. The visualization of protein mass diffusion process has generally technical difficulties because the event of protein transport takes on slow and small phenomenon. There is a great lack of reliable experimental data due to the measurement difficulties encountered when measuring a process as slow as transport phenomena. The technique proposed in this study solves this problem and a series of clear visualized images of concentration profiles of hindered diffusion field was obtained. From the results, it is considered that the active mass flux control of protein can be realized by changing the macropore patterning and pore diameter on the separated membrane. The capability and technique for precise control of protein mass transfer are discussed.
Biography
Professor Atsuki Komiya is head of Heat Transfer Control Laboratory in the Institute of Fluid Science, Tohoku University, Japan. He received the PhD in mechanical engineering in Tohoku University. From 2002 to 2004, he was a Research Fellow with the Japan Aerospace Exploration Agency (JAXA). He worked the development of the facility of fluid experiment for space experiment. In 2004, he moved to the Tohoku University as an Assistant Professor. Since 2019, he has been a Professor of Tohoku University. He is the author of two books, more than 140 refereed papers. Professor Komiya’s awards and honors include the Young Researcher Award and Scientific Contribution Award of the Heat Transfer Society of Japan, and the Young Scientists’ Prize of the Commendation for Science and Technology by the Minister of Education, Culture, Sports, Science and Technology.
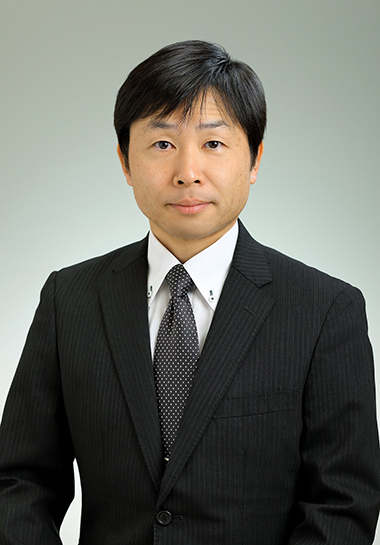
Invited Talk
When Surfaces Matter: Using Nano-scaled Surface Features to Control Macro-scale Transport
Professor Gary Rosengarten
School of Mechanical and Automotive Engineering, RMIT University
Typical heat transfer correlations do not consider the effect of surface properties for processes such as convection or radiation. However, even nano-scaled features can have a massive effect on transport properties. In this presentation I will show some of our work demonstrating how micro- and nano-scale engineering can be used to control and enhance both heat and mass transport.
Biography
Professor Gary Rosengarten is head of the Laboratory for Innovative Fluid Thermal Systems (LIFTS) in the School of Engineering at RMIT University and leader of the Energy Cluster at RMIT. Prior to joining RMIT University in 2012, he spent 6 years at UNSW running the heat transfer group, and being head of the thermal fluids research area. He also has 2 years experience in consulting for sustainable building design. He has first class honours degrees in Mechanical Engineering and in Physics from Monash University, and a PhD in Mechanical Engineering from the University of NSW. He won the inaugural American Society of Mechanical Engineers (ASME) Solar Energy Division Graduate Student award in 2000. In the last 6 years he has been awarded over $6 million in funding from ARENA for various renewable energy project. He has approximately 150 refereed papers in fields ranging from Solar Energy to Biotechnology.
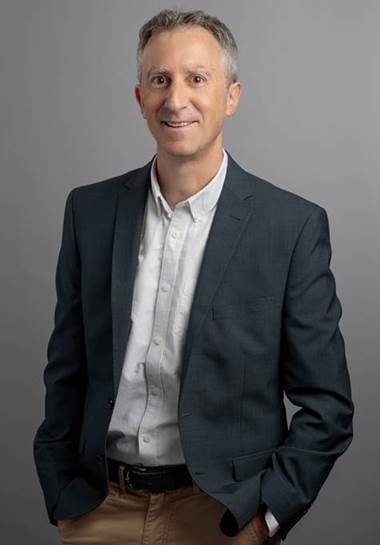
Invited Talk
Hybrid Lattice Boltzmann method for viscoelastic fluid flow instabilities and elastic turbulence
Associate Professor Emilie Sauret
School of Mechanical, Medical & Process Engineering, Queensland University of Technology
Viscoelastic fluids, a subclass of non-Newtonian fluids in which polymers are added to a solvent, exhibit interesting nonlinear material properties, which offer a range of exciting practical benefits. It is well-known that in the absence of inertial effects (Reynolds number ≤ 1) these viscoelastic fluids generate an anisotropic elastic stress contribution that transitions the flow to a novel chaotic regime, known as elastic turbulence (ET). This purely elastic instability shares a lot of features with traditional inertial turbulence, namely, (i) increased flow resistance, (ii) enhanced mixing, (iii) random flow fluctuations characterized by a broadband spectrum. As a result, viscoelastic instabilities, specifically ET, have naturally emerged as an obvious solution to the long-encountered mixing challenges in microfluidics, as well as further enhancing the heat transfer process in micro-cooling technologies.
Despite these various exciting benefits, the numerical difficulties associated with simulating viscoelastic instabilities have left a lot still unexplored. Recently, methods based on the lattice Boltzmann method (LBM) have emerged as a viable numerical tool for studying the behaviour of viscoelastic fluids. However, the inability to preserve numerical stability, especially at high elastic effects have restricted previous LBM attempts from simulating viscoelastic instabilities.
In this talk, I will present the capabilities of a hybrid LBM model, capable of overcoming previously encountered stability issues when simulating viscoelastic instabilities. The model is subsequently applied to simulate ET for which I will demonstrate that the global artificial stress diffusivity term commonly introduced in rheological models to enhance numerical stability can introduce unphysical artifacts, resulting in a rapid loss of physical meaningfulness. To conclude, I will present a new modified artificial diffusivity approach. By limiting artificial diffusivity only to regions likely to encounter stability issues. I will demonstrate that this modified approach is devoid of unphysical artifacts, allowing all features of ET to be retained.
Ultimately, this work proves the capability of applying a coupled LBM with modified artificial diffusivity to accurately simulate the complex flow behaviour of viscoelastic instabilities, specifically ET, advancing the capability to explore more practical and challenging cases.
Biography
Dr. Emilie Sauret is currently Associate Professor in the School of Mechanical, Medical & Process Engineering, Queensland University of Technology (QUT), and an elected council member of the Australasian Fluid Mechanics Society. She received her PhD degree in Turbulence Modelling from the University Pierre & Marie Curie, Paris, France in 2004. Prior to her postdoctoral position (2009-2012) at the University of Queensland, she spent 5 years in the automotive and oil and gas industry both in France and in Australia. In 2013, she was awarded an ARC-DECRA and joined QUT where she teaches in the Mechanical Engineering degree. Dr. Sauret has extensive interdisciplinary research experience in computational fluid dynamics, applied mathematics and applied physics. Her current research focusses on the development of advanced computational techniques to accurately simulate complex non-ideal fluid flow behaviours that are critical for the rational design and robust optimisation of engineering applications, in particular in the field of energy and biomedical engineering. She has produced over 80 publications, attracted over $8M in research funding and established collaborations across the globe. In 2019, an Endeavour Leadership Fellowship supported her visiting position at MIT and in 2020, Dr. Sauret was awarded an ARC-Future Fellowship to uncover fundamental microscale physics, pioneering research on computational microfluidics.
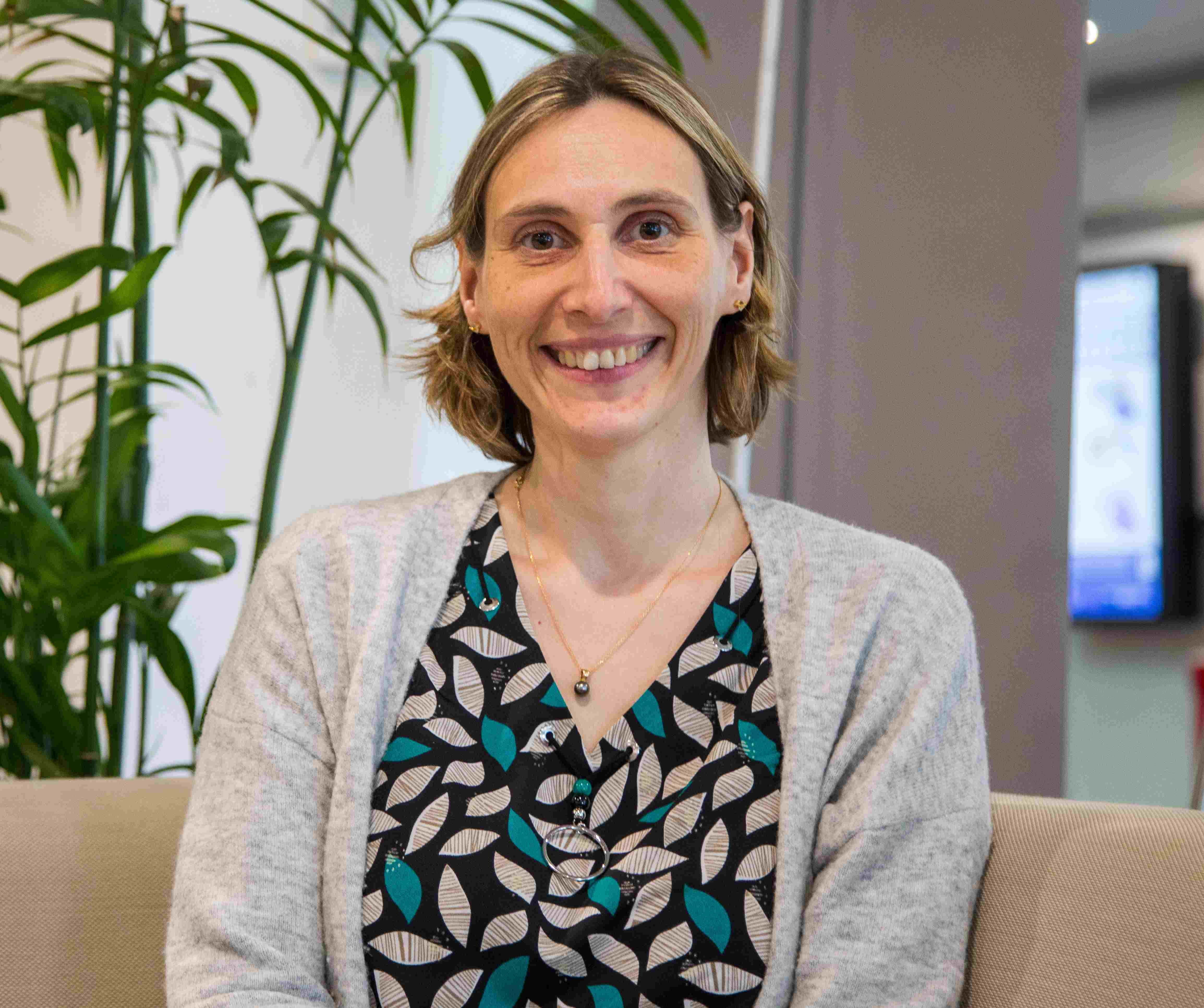
Invited Talk
Turbulent Vertical Natural Convection Boundary Layers – Insights Gained from DNS up to Grd = 1.8 x 108
Dr Nicholas Williamson
School of Aerospace, Mechanical & Mechatronic Engineering, The University of Sydney
The natural convection boundary layers (NCBLs) that form adjacent to a vertical heated surface occur widely in engineering problems and also in the natural environment. Some of these applications occur at very large scales and at Grashof numbers (Gr) which significantly exceed the conditions obtained by laboratory experiments or DNS to date. This talk will focus on the mechanics of these large scale turbulent flows and recent observations made using DNS of temporally evolving parallel NCBL flow up to Grd = 1.8×108 at Pr = 0.71 (Grx ~ 2.6 x 1011). At high Gr, this flow is comprised of a turbulent boundary layer region that is coupled with a turbulent outer plume. The DNS show that the boundary layer exists within a constant forcing layer and suggest that direct contributions of buoyancy to this forcing remain significant until Grd > 109. Within this range, the direct action of buoyancy leads to modification of the turbulent log-law of the wall for the mean velocity profile, the form of which will be discussed. The outer bulk plume-like region is shown to attain self-similar Gr-independent behaviour after Grd = 107 and the surface heat transfer and shear are directly related to the top-hat scales which characterise the plume-like flow. The heat transfer at Grd = 1.8 x 108 is shown to attain characteristics of the ultimate flow regime, with Nu ~ Gr0.37 in comparison with Nu ~ Gr0.33 seen in laboratory studies at lower Gr. The high Gr scaling is analogous to that identified for Rayleigh Bernard (Grossman and Lohse, 2000; 2011). The transition to and characteristics of this regime will be discussed.
Dr Nicholas Williamson is a Senior Lecturer in the School of Aerospace, Mechanical and Mechatronic Engineering at the University of Sydney. His research focuses on buoyant turbulent flows, in particular natural convection boundary layers and free shear flows such as negatively buoyant jets. He also works collaboratively in riverine science towards understanding the physical mechanisms which cause algal blooms and how stable thermal stratification aids bloom formation.
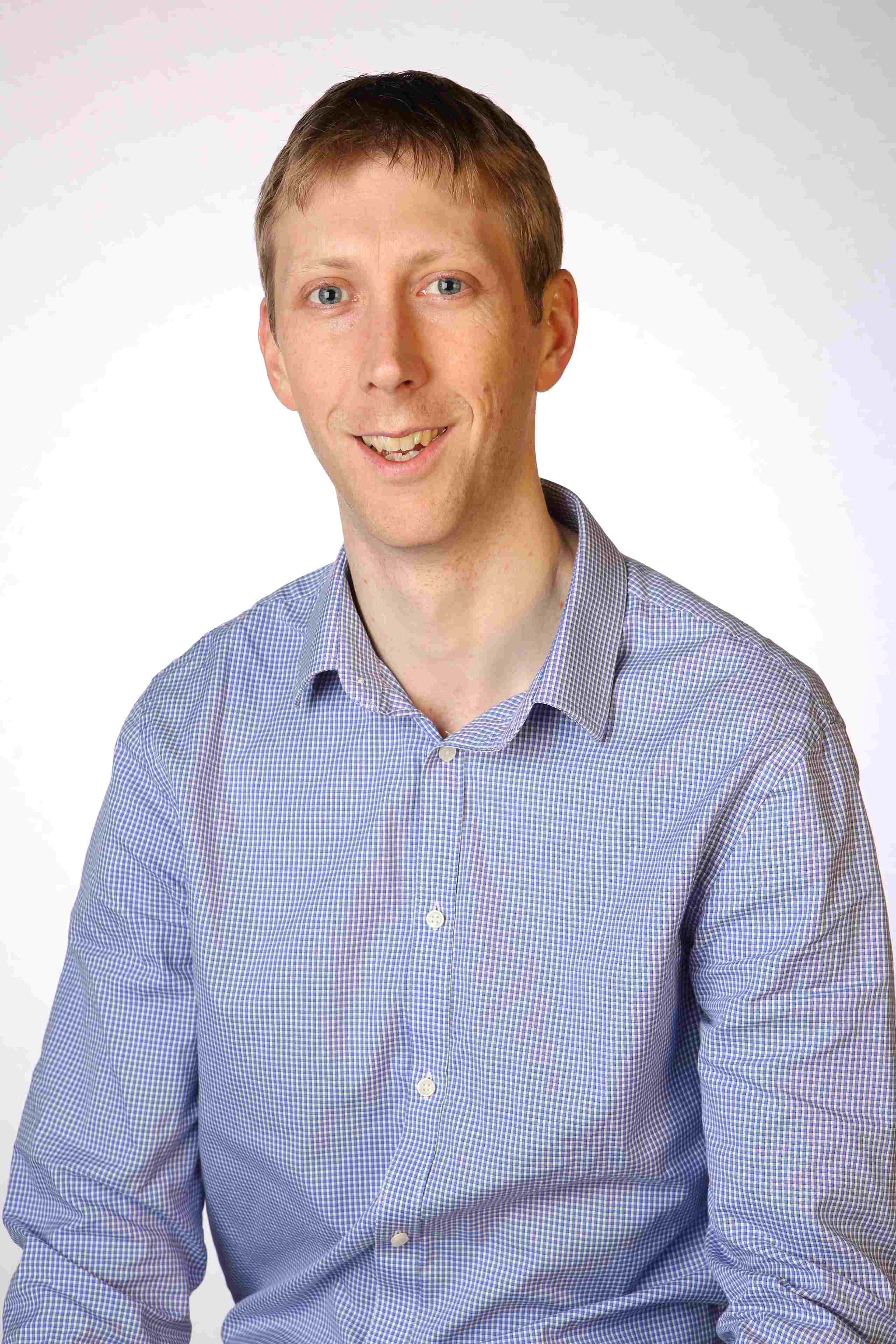